 |
Komatiites
and the Plume Debate
|
|
Photo
of komatiite outcrop in the type section (Komati
formation) in the Barberton Mountainland, South
Africa |
|
Stephen
Parman
Experimental Petrology Laboratory,
Massachusetts Institute of Technology, Cambridge, MA 02139, USA
parman@MIT.EDU
|
Click here
to go to Discussion of this page |
Introduction
Large igneous provinces (LIPs) and ocean islands basalts
(OIB) are commonly hypothesized to be the products of thermal plumes
originating at the core-mantle boundary. If so, then the size, strength
and number of such mantle upwellings should primarily be a function
of the temperature of the core (Davies, 1993). Thus one way
to test the plume hypothesis is to look at the size and frequency of
such proposed plumes throughout the history of the Earth. Numerical
simulations of mantle convection and the cooling of the Earth typically
show a rapid cooling in the first 1 billion years, followed by a slower,
nearly linear cooling (Davies, 1993). Correspondingly, one
would expect a steep decline in plume activity in the first 1 b.y. of
Earth history. Unfortunately, the Archean and Hadean rock record is
very scant. Furthermore, most modern proposed plume magmas erupt on
oceanic crust, which only very rarely escapes destruction at convergent
margins. It seems likely that such size-frequency information on mantle
plumes simply has not been preserved in the rock record.
The strength (buoyancy flux) of the hypothetical plumes
is another matter. Buoyancy flux is primarily a function of the temperature
difference between the upwelling mantle and the ambient mantle. If one
constrains the ambient mantle to be below (or at least near) its solidus
(i.e. no magma ocean after ~ 4.4 Ga) then the primary influence on the
strength of thermal plumes will be the temperature of the plume when
it leaves the CMB. Studies (mostly of mid-ocean ridge volcanism) have
shown that the temperature of a rising piece of mantle that undergoes
anhydrous, adiabatic decompression melting can be correlated with the
major element composition of the magmas it produces (Klein &
Langmuir, 1987). Hotter mantle intersects its solidus at greater
depths and melts over a larger pressure interval and to greater extents
than cooler mantle. The melts produced will have higher MgO and FeO
and lower Na2O, Al2O3 and SiO2
than melts of cooler mantle. So an alternative approach to quantifying
the vigor of plume activity through time is to look at the secular evolution
of the major element composition of magmas thought to be produced by
plumes.
Like the cooling of the Earth, one would expect the
most rapid changes and dramatic differences in plume magma chemistry
to occur in the first billion years of Earth history. Indeed, studies
of Archean volcanism (Arndt, 1976; Pyke et al., 1973;
Viljoen & Viljoen, 1969a,b) have found high-MgO magmas
that seem to fit the criteria for being the products of super-hot plumes
(Herzberg, 1995). These magmas are called komatiites. Recently
though, an old debate (Allegre, 1982) has been rekindled that
argues these magmas are not produced by plumes, but rather by hydrous
melting processes in the upper mantle (Parman & Grove,
2001).
Komatiites
Komatiites were first recognized in the late
1960s in the Barberton Mountainland greenstone
belt in South Africa (Viljoen & Viljoen,
1969a,b). They have extremely high MgO contents;
18-30 wt.% compared to 10-15 wt.% for the
most mafic mid-ocean ridge basalts (MORB)
or ocean-island basalts (OIB, Figure 1). The
MgO contents of magmas is proportional to
their melting temperatures (higher MgO means
hotter magmas) and the first experiments on
komatiites (Green, 1975) were interpreted
to imply melting conditions in excess of 1600°C
(compared to 1250-1350°C for modern MORB,
Figure 2; see also Temperature
and Mantle temperature
pages). Subsequent dating showed the Barberton
komatiites to be 3.5 billion years old (Lopez-Martinez
et al., 1992) and so the high temperatures
inferred for the komatiite source region fit
nicely with the concept of a hot early Earth.
Komatiites from the Superior province in
Canada (the Munro komatiites) were the next
to be well studied (Arndt, 1976;
Pyke et al., 1973). These are younger
(2.7 Ga) than the Barberton komatiites and
have lower MgO contents (up to ~ 24 wt.%).
This also fit well with the idea of a cooling
Earth. At the time, there was still some debate
about the tectonic setting of komatiites.
A whole range of settings was considered,
e.g., mid-ocean ridge, plume, giant
impact and magma oceans.
|
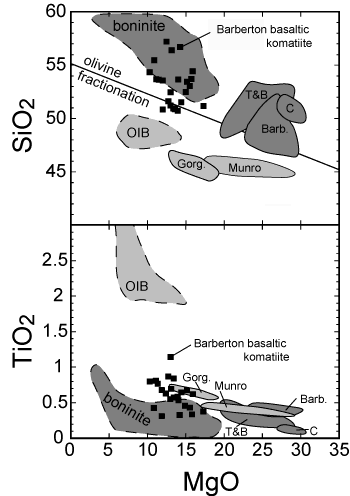
|
Figure 1: SiO2
and TiO2
versus MgO in komatiites (fields with solid
boundaries), basaltic komatiites (filled squares)
and modern mafic magmas (fields with dashed
boundaries). Solid line in top panel shows compositional
effect that olivine fractionation would have
on the most MgO-rich Barberton komatiite. The
majority of the basaltic Barberton komatiites
cannot be made by olivine fractionation from
a komatiite parent. Boninites (modern subduction
related magmas) show a large compositional overlap
with Archean basaltic komatiites. Komatiites
show a wide range of major and minor element
composition. High-SiO2
komatiites (dark fields) resemble modern boninite
magmas that are produced by hydrous melting,
while low-SiO2
komatiites (light fields) resemble more closely
modern basalts produced by anhydrous decompression
melting. The low TiO2
contents of komatiite magmas requires their
source to have been depleted (i.e. to already
have had a melt extracted). Komatiites: Barb.
(Barberton, South Africa, 3.5 Ga,), C (Commondale,
South Africa, 3.3 Ga), T (Tisdale, Canada, 2.7
Ga), B (Ball, Canada, 2.9 Ga), Munro (Munro,
Canada, 2.7 Ga) and Gorg. (Gorgona, South America,
0.088 Ga). Modern magmas: OIB (ocean island
basalt, GeoRoc online database), boninites (GeoRoc
online database). |
The Plume Model
It is not clear at what point plumes
became the paradigm for the generation of komatiites.
One important step was the discovery of Phanerozoic
komatiites on the island of Gorgona (Aitken &
Echeverria, 1984; Echeverria, 1980).
These komatiites have even lower MgO and melting temperatures
than the Munro komatiites, again fitting nicely into
the cooling Earth model (Figure 2). Initially, the
tectonic setting of Gorgona was unclear. The main
issue was whether it is related to the orogenies on
the mainland of the South American continent or to
the Galapagos hotspot. Though I have not seen the
resolution of this debate, more recent papers on Gorgona
have assumed that it is related to a plume beneath
the Galapagos, thus cementing the idea that komatiites
are produced by plumes (Arndt et al., 1998;
Echeverria & Aitken, 1986). Another benchmark
in the study of komatiites was the publication of
the book “Komatiites” (Arndt
& Nisbet, 1982), which summarized much of
what was then known about komatiites. The general
consensus of the book is that komatiites are produced
by plumes.
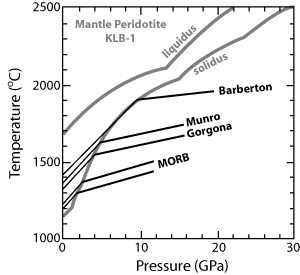
|
The
basic concepts of the plume model are summarized
in Figure 2 and follow fairly closely the models
of adiabatic, decompression melting developed
to explain melting at mid-ocean ridges (Klein
& Langmuir, 1987). Barberton komatiites
(3.5 Ga) had the hottest mantle sources. As
the Barberton plume ascended, the mantle intersected
the solidus at depths greater than 350 km (Walter,
1998). Melting occurs between the point of intersection
with the solidus and the bottom of the lithosphere,
perhaps 50-100 km thick in the Archean. Assuming
~1 percent melting per 3 km of ascent when cpx
is present and half that after it has been all
melted out, 60-70% melting is implied. By 2.7
Ga, the mantle has cooled considerably, and
now the Munro plume adiabat crosses the solidus
at much shallower depths, ~200 km, and produces
30-40% melting. Gorgona komatiites are cooler
still and begin melting at ~150 km with melt
extents of 20 – 30%. Most komatiites have
been shown to have source regions that were
depleted by a previous melt extraction event,
and so the actual amount of melting that produced
the komatiites is probably lower than the numbers
given above. |
Figure
2: Depths and temperatures of melting of komatiites
in a plume setting inferred by Herzberg (1995).
Black lines show the pressure-temperature path
experienced by a body of mantle as it ascends
adiabatically towards the surface. The gray
lines are the solidus and liquidus of KLB-1,
a fertile mantle composition. The solidus is
the first point at which the mantle begins melting.
The liquidus is the point at which the mantle
is completely molten. Below the solidus, the
slope of the lines reflects slight cooling due
to decompression. The potential temperature
is the temperature at which this path intersects
the surface. The greater slope above the solidus
reflects both the heat lost to produce melting
and greater compressibility of melts relative
to solid. In the plume model, the oldest komatiites
(Barberton at 3.5 Ga) record the highest mantle
potential temperatures, while late Archean (2.7
Ga) Munro komatiites and Cretaceous (8.8 Ma)
Gorgona komatiites are formed in progressively
cooler plumes. Note that if melting occurs by
adiabatic decompression, then higher melting
temperatures require that melting begins at
greater depths. The range of melting temperatures
for present day mid-ocean ridge basalts (MORB)
is also shown. |
The Subduction model
Along the way, as with all paradigms right or wrong,
there were dissenting voices. Brooks & Hart (1972; 1974)
first pointed out that the major-element chemistry of many of the komatiites
and related magmas (komatiitic basalts) were more similar to modern
mafic subduction magmas than to any magma thought to be produced by
a modern plume (Gorgona had not been discovered at this point). Allegre
et al. (1982) pointed out that the very high melting temperatures
could be lowered if the melting incorporated significant amounts of
H2O. Perhaps the first strong evidence for a subduction origin
for a suite of komatiites was presented by Wilson & Versfeld
(1994) who studied the Nondweni komatiites. These komatiites have much
higher SiO2 than the Munro or Gorgona komatiites, and show
some similarities to modern mafic subduction magmas (boninites, Figure
1). SiO2 contents of magmas generally decrease as the pressure
of melting increases, and so high SiO2 is difficult to explain
in a plume scenario. Boninites have similarly high SiO2 at
high MgO contents because they are high-degree melts produced at shallow
depths. In an anhydrous, decompression melting regime, as plumes are
thought to represent, high degrees of melting necessarily must begin
at great depth, but in the case of boninites, large extents of melting
can occur at shallow depth because the melting is caused by high H2O
contents (Crawford et al., 1989). While Wilson & Versfeld
(1994) noted the similarities with boninites, they stopped short of
arguing for a subduction origin.
The first evidence for high H2O contents
came from Stone et al. (1997) who discovered hydrous amphibole
in a komatiite. Most komatiites contain hydrous tremolite, but this
is produced by the metamorphism that has affected all komatiites and
is not indicative of the H2O content of the magma. Stone
et al. (1997) found pargasitic amphibole which is often an igneous
mineral though it can also be produced by metamorphism. Hanski
(1992) also found pargasitic amphibole in Fe-rich komatiites in Finland.
Melt inclusions, now devitrified and possibly altered, have also been
analyzed for H2O contents (McDonough & Ireland,
1993; Shimizu et al., 2001) with up to 0.8 wt.% water being
inferred.
I entered komatiite research as graduate student in Tim Grove’s
lab at MIT. Maarten de Wit had shown Tim some work he was doing
on the Barberton komatiites and Tim noted that the igneous clinopyroxene
that was sometimes preserved in the rocks was fairly high in
Ca (wollastonite component) given the low CaO contents of the
magmas. Having worked extensively with hydrous, mafic magmas,
Tim knew that one effect of H2O was to produce high-Ca
pyroxene.
So my task was set; run dry experiments and see if I could
reproduce the high Ca clinopyroxene (augite) found in the Barberton
komatiites. Needless to say, it didn’t work. Under dry
conditions, the first pyroxene to appear was actually pigeonite
(low Ca cpx), which is not surprising given the ultramafic composition
of the komatiites (Parman et al., 1997). The augite
that subsequently crystallized was significantly lower in CaO
(Wo = 0.3 – 0.35) than found in the rocks (Wo = 0.4 –
0.43). This is true whether the augite is crystallized under
isothermal or cooling conditions.
However, when ~ 6 wt.% H2O was added to the melt,
the pigeonite was suppressed and the first pyroxene to crystallize
was augite (as is the case in the rocks). Even better, the compositions
of the experimental augites were an excellent match for the
natural augites (Figure 3). Correcting for olivine crystallization,
the data suggest 3 – 4 wt.% H2O in the Barberton
komatiite melts.
|
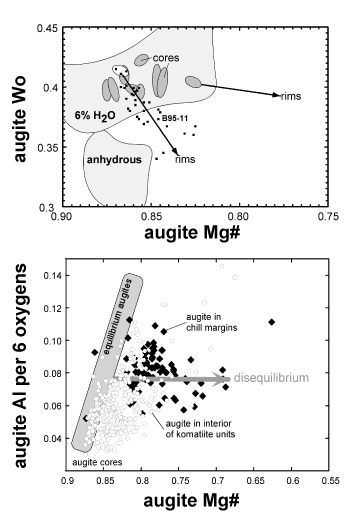
Figure 3: Composition of augite in Barberton
komatiites and in dry and wet experiments. Top: Augites produced
in anhydrous experiments (labeled field) have distinctly lower
wollastonite (Wo) contents than the cores of augites found in
the komatiites (darker fields), while augite in experiments with
6 wt.% H2O
are a good match for the natural augite (labeled field). Points
show the compositional zoning from core (high Mg#) to rim (low
Mg#). Mg# = Mg/(Mg+Fe). Bottom: Augites in komatiites that grew
near the chill margin (diamonds) and so grew under rapid cooling
conditions incorporated high Al contents, while the augite that
grew in the interior (circles; and cores in 4a) are close to the
equilibrium values measured in experiments, indicating that kinetics
have had little effect on the major-element composition of the
augites. |
At first, it was unclear whether the H2O
required the Barberton komatiites to be produced in a subduction zone,
or whether a hydrous plume scenario was possible. Subsequently, we compared
the major and trace element chemistry of komatiites and basaltic komatiites
with modern mafic magmas (Parman et al., 2001; Parman et
al., 2003). As had been found before (Cameron et al.,
1979), the compositions of the basaltic komatiites is very similar to
modern boninites (Figures 1 and 4b). Small differences are: slightly
higher TiO2 and FeO, smaller high-field-strength element
(HFSE = Nb, Ta, Zr, Hf, Ti) anomalies, and less depletion in middle
rare-earth elements (REE). Nevertheless, basaltic komatiites are much,
much closer to boninites than to any OIB or supposedly plume related
picrite (Figure 1). Many are indistinguishable from modern boninites.
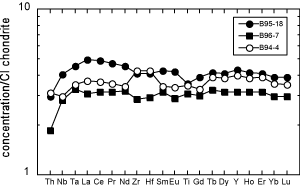
Figure 4a: Trace-element composition of Barberton komatiites.
Two samples from Barberton show HFSE depletions, while the third
(B94-4) has the same unusual high Zr seen in boninite and basaltic
komatiites (Figure 2). B94-4 also has a slightly U-shaped REE
pattern.
|
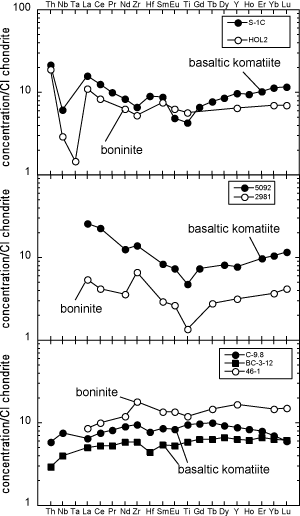
Figure 4b: Comparison of the trace-element composition
of Barberton basaltic komatiites with Phanerozoic boninites.
Elements arranged in order of compatibility. Both boninites
and basaltic komatiites show a wide range of LREE slopes, sometimes
resulting in U-shaped patterns. HFSE are variable, sometimes
showing large depletions and unusual Zr enrichments.
|
Komatiites are higher in MgO than boninites,
and one cannot make a direct comparison. However, there are similarities.
High SiO2 for given MgO, very low TiO2 (Figure
1), variable LREE (Figure 4a) and in particular an interesting
decoupling between Zr-Hf and Ti. Usually these HFSE behave similarly,
but in some komatiites (and many modern boninites), Zr and Hf
are enriched relative to the REE (e.g., sample B94-4
in Figure 4b) while Ti is depleted. How this is produced in boninites,
let alone the komatiites, is not clear, but it is a very uncommon
feature of other types of magmas and has been used as diagnostic
feature of boninites (Hickey & Frey, 1982). |
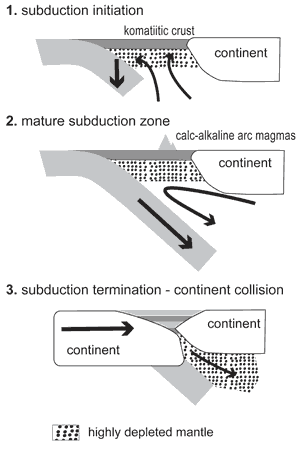
Figure 5: Subduction model for the origin of komatiites
in subduction zones, modeled after melting process inferred
for boninites (Stern & Bloomer, 1992). 1. Boninite-like
melting event produces a range of high-MgO melts: komatiite,
komatiitic basaltic magmas and low-Ti tholeiites (dark shading)
and a corresponding heavily depleted mantle residue (stippled
region). 2. Continued subduction of the lithosphere cools the
mantle and establishes a mature subduction. Subsequent lower
temperature hydrous magmas (calc-alkaline andesites) are emplaced
on top of early, ultra-mafic crust. The cold, buoyant residual
mantle remains beneath the fore-arc. 3. Komatiitic crust is
obducted onto continents during continent collision at the end
of subduction. The depleted mantle is thickened and incorporated
into the continental lithospheric mantle.
|
The chemical similarities between boninites, basaltic komatiites
and komatiites has led us to the conclusion that komatiites
were produced by similar melting processes that produce modern
boninites, with the primary difference being that the mantle
was about 100°C hotter in the Archean (Parman et al.,
2001). This estimate is based on high-pressure, H2O-undersaturated
melting experiments (Parman & Grove, 2004). Of
course, the origin of boninites themselves is a matter of some
debate. For simplicity, we have focused on the model that seems
most plausible to us (Stern & Bloomer, 1992) in
which boninites are formed at the initiation of subduction (Figure
5). Other models suggest subduction of mid-ocean ridges or the
influence of plumes leads to the production of boninites (Crawford
et al., 1989) and it is seems likely that boninites are
formed by various arc-related melting processes. But in all
cases, the inferred melting conditions (shallow, hydrous melting)
are the same and do not change the ultimate conclusion that
production of komatiites by hydrous melting would require a
slightly (~ 100°C) hotter upper mantle in the Archean.
Subsequent to the publication of our model, Wilson discovered
another high-SiO2 komatiite location – Commondale
(Wilson, 2003; Wilson et al., 2003). These
have over 50 wt.% SiO2 and trace element contents
that suggest a very depleted mantle source. They are the most
boninitic of all komatiites in major element composition and
source depletion. Unlike most boninites, their LREE and LILE
have not been enriched by the hydrous fluid that is hypothesized
to have instigated the melting event. On the other hand, there
are a number of boninites that are not LILE enriched (Figure
4b). Wilson and colleagues conclude that both of these characteristics
are difficult to reconcile with a plume origin, and require
some type of hydrous melting process, probably subduction.
|
Final Thoughts
A trend that has become clear in the last few years
is that advocates of a subduction origin for komatiites focus on the
high-SiO2 komatiites e.g., Commondale, Nondweni
and Barberton, while plume advocates focus on low SiO2, OIB-like
komatiites e.g., Munro and Gorgona. This may suggest that komatiites
were produced in more than one tectonic setting, though personally I
find this unappealing. The similarities in composition and geology of
komatiites seem to me to be much greater than their differences. To
reconcile the two opposing hypotheses, some researchers have proposed
hybrid models in which komatiites are produced by hydrous melting in
Archean plumes (Asahara & Ohtani, 2001). The water is envisioned
to be primordial H2O inherited from the accretion of the
planet.
Whatever one's view on komatiites, I think it is fair
to say that their origins are the subject of active debate. Furthermore,
there are some komatiites, Commondale in particular, that are very difficult
to fit into a plume scenario because their high SiO2 suggests
relatively shallow melting. If it is eventually shown that all (or most)
komatiites were produced by subduction-related melting processes, this
would call into question the presence of thermal mantle plumes in the
modern world. Komatiites, as the product of super-hot Archean plumes,
are an important stone in the foundation of plume theory in general.
If komatiites are not produced in plumes, then there is no evidence
for great plume activity in the early Earth, even though this is a fundamental
prediction of mantle convection models that incorporate thermal plumes.
Then, views of thermal plumes in the modern mantle are also called into
question or, at least, need to be modified substantially. Conversely,
if modern OIB e.g., at Hawaii are indeed the product of upper
mantle melting, and not of thermal plumes from the CMB, this also calls
into question the origin of komatiites in thermal plumes.
|
-
Aitken B. G. and Echeverria L. M. (1984) Petrology
and geochemistry of komatiites and tholeiites from Gorgona Island,
Colombia. Contributions to Mineralogy and Petrology 86,
94-105.
-
Allegre C. J., (1982) Genesis of Archaean komatiites
in a wet ultramafic subducted plate. In: Arndt N. T., and Nisbet E.
G. (Eds.) Komatiites. Springer-Verlag, Berlin, pp. 495-500.
-
Arndt N. and Nisbet E.G. (1982)
Komatiites. Allen and Unwin, London, p.
526.
-
Arndt N., Ginibre C., Chauvel C., Albarede F.,
Cheadle M., Herzberg C., Jenner G., and Lahaye Y. (1998) Were komatiites
wet? Geology 26, 739-742.
-
Arndt N. T. (1976) Ultramafic Lavas (Komatiites)
from Munro Township, Ontario. Transactions-American Geophysical
Union 57, 355-355.
-
Asahara Y. and Ohtani E. (2001) Melting relations
of the hydrous primitive mantle in the CMAS- H2O system
at high pressures and temperatures, and implications for generation
of komatiites. Physics of the Earth and Planetary Interiors
125, 31-44.
-
Brooks C. and Hart S. (1972) An Extrusive Basaltic
Komatiite from a Canadian Archean Metavolcanic Belt. Canadian
Journal of Earth Sciences 9, 1250-1253.
-
Brooks C. and Hart S. R. (1974) On the significance
of komatiite. Geology 2, 107-110.
-
Cameron W. E., Nisbet E. G., and Dietrich V. J.
(1979) Boninites, komatiites and ophiolitic basalts. Nature.
280, 550-553.
-
Crawford A J., Falloon T J. and Green D.H. (1989)
Classification, petrogenesis and tectonic setting of boninites. In:
Crawford A.J. (Ed.) Boninites. Unwin Hyman, London, pp.1-49.
-
Davies G. F. (1993) Cooling the Core and Mantle
by Plume and Plate Flows. Geophysical Journal International
115, 132-146.
-
Echeverria L. M. (1980) Tertiary or Mesozoic komatiites
from Gorgona Island, Colombia; field relations and geochemistry. Contributions
to Mineralogy and Petrology 73, 253-266.
-
Echeverria L. M. and Aitken B. G. (1986) Pyroclastic
rocks; another manifestation of ultramafic volcanism on Gorgona Island,
Colombia. Contributions to Mineralogy and Petrology 92,
428-436.
-
Green D. H. (1975) Genesis of Archean Peridotitic
Magmas and Constraints on Archean Geothermal Gradients and Tectonics.
Geology (Boulder) 3, 15-18.
-
Hanski E. J. (1992) Petrology of the Pechenga ferropicrites
and cogenetic, Ni-bearing gabbro-wehrlite intrusions, Kola Peninsula,
Russia. Bulletin - Geological Survey of Finland 367,
192.
-
Herzberg C. (1995) Generation of Plume Magmas through
Time – an Experimental Perspective. Chemical Geology
126, 1-16.
-
Hickey R. L. and Frey F. A. (1982) Geochemical
characteristics of boninite series volcanics; implications for their
source. Geochimica et Cosmochimica Acta 46,
2099-2116.
-
Klein E. M. and Langmuir C. H. (1987) Global Correlations
of Ocean Ridge Basalt Chemistry with Axial Depth and Crustal Thickness.
Journal of Geophysical Research 92, 8089-8115.
-
Lopez-Martinez M., York D., and Hanes J. A. (1992)
A Ar-40/Ar-39 Geochronological Study of Komatiites and Komatiitic
Basalts from the Lower Onverwacht Volcanics – Barberton Mountain
Land, South-Africa. Precambrian Research 57,
91-119.
-
McDonough W. F. and Ireland T. R. (1993) Intraplate
Origin of Komatiites Inferred from Trace-Elements in Glass Inclusions.
Nature 365, 432-434.
-
Parman S. W., Dann J. C., Grove T. L., and deWit
M. J. (1997) Emplacement conditions of komatiite magmas from the 3.49
Ga Komati Formation, Barberton Greenstone Belt, South Africa. Earth
and Planetary Science Letters 150, 303-323.
-
Parman S.W. and Grove T. L. (2004) Harzburgite
melting with and without H2O: Experimental data and predictive
modeling. Journal of Geophysical Research 109,
doi:10.1029/2003JB002566.
-
Parman S. W., Grove T. L., and Dann J. C. (2001)
The production of Barberton komatiites in an Archean subduction zone.
Geophysical Research Letters 28, 2513-2516.
-
Parman S. W., Shimizu N., Grove T. L., and Dann
J. C. (2003) Constraints on the pre-metamorphic trace element composition
of Barberton komatiites from ion probe analyses of preserved clinopyroxene.
Contributions to Mineralogy and Petrology 144,
383-396.
-
Pyke D. R., Naldrett A. J., and Eckstran.Or. (1973)
Archean Ultramafic Flows in Munro Township, Ontario. Geological
Society of America Bulletin 84, 955-977.
-
Shimizu K., Komiya T., Hirose K., Shimizu N., and
Maruyama S. (2001) Cr-spinel, an excellent micro-container for retaining
primitive melts – implications for a hydrous plume origin for
komatiites. Earth and Planetary Science Letters 189,
177-188.
-
Stern R. J. and Bloomer S. H. (1992) Subduction
zone infancy; examples from the Eocene Izu-Bonin-Mariana and Jurassic
California arcs. Geological Society of America Bulletin 104,
1621-1636.
-
Stone W. E., Deloule E., Larson M. S., and Lesher
C. M. (1997) Evidence for hydrous high-MgO melts in the Precambrian.
Geology 25, 143-146.
-
Viljoen M. J. and Viljoen R. P. (1969a) Evidence
for the existence of a mobile extrusive peridotitic magma from the
Komati Formation of the Onverwacht Group, Upper Mantle Project.
Special Publication – Geological Society of South Africa
2, 87-112.
-
Viljoen M. J. and Viljoen R. P. (1969b) The geology
and geochemistry of the Lower Ultramafic Unit of the Onverwacht Group
and a proposed new class of igneous rocks, Upper Mantle Project.
Special Publication – Geological Society of South Africa
2, 55-85.
-
Walter M. J. (1998) Melting of garnet peridotite
and the origin of komatiite and depleted lithosphere. Journal
of Petrology 39, 29-60.
-
Wilson A. H. (2003) A new class of silica enriched,
highly depleted komatiites in the southern Kaapvaal Craton, South
Africa. Precambrian Research 127, 125-141.
-
Wilson A. H., Shirey S. B., and Carlson R. W. (2003)
Archaean ultra-depleted komatiites formed by hydrous melting of cratonic
mantle. Nature 423, 858-861.
-
Wilson A. H. and Versfeld J. A. (1994) The early
Archaean Nondweni greenstone belt, southern Kaapvaal Craton, South
Africa; Part II, Characteristics of the volcanic rocks and constraints
on magma genesis. Precambrian Research 67,
277-320.
|
Discussion
Monday June 28th, 2004: Gillian Foulger
You write “Furthermore, most modern proposed plume magmas
erupt on oceanic crust, which only very rarely escapes destruction at
convergent margins. It seems likely that such size-frequency information
on mantle plumes simply has not been preserved in the rock record.”
There is a school of though that oceanic plateaus are not subducted,
of course, so presumably they all ought to be accreted to continents.
Monday June 28th, 2004: Steve Parman
The unsubductable plateau theory is interesting. I have no strong feeling
one way or the other, but to my knowledge there are only two or three
clear cases in the entire continental rock record of an accreted plateau
(Wranglia in N. America and one in the Himalayas). If they cannot be
subducted, I would expect a lot more.
Friday July 9th, 2004: Don Anderson
Dear Steven, Your komatiite contribution is an excellent summary of
the current debate. It is also accessible to the non-specialists who
have been told that komatiites in the Archean prove the existance of
plumes today! There are two minor missing points that complete your
story.
The first is the complete overlap of the isotopic signatures
of komatiites with MORB (e.g., Anderson, Don L., 1994, Komatiites
and picrites: Evidence for a depleted “plume” source, Earth
and Planetary Science Letters, 128, 303-311).
That is, the highest temperature magmas are not OIB-like, they are MORB-like!
The depleted nature of MORB is often taken as evidence that they come
from the upper mantle and, in fact, are representative of the whole
upper mantle.
The second issue is the evidence that the Earth has
cooled considerably since the Archean, meaning that high temperatures
for Archean magmas are expected. The evidence usually quoted against
such cooling (inferred temperatures of cratonic keels, the inferred
“Archean catastrophe” if current cooling rates are extrapolated
backwards) have found solutions (Korenaga, J., 2003, Energetics of mantle
convection and the fate of fossil heat, Geophys. Res. Lett.,
30, 1437, doi:10.1029/2002GL016179, 2003; Lenardic,
A., 1998, On the partitioning of mantle heat loss below oceans and continents
over time and its relationship to the Archean paradox, Geophys.
J. Int., 134, 706-720).
This is not to say that some komatiites are not particularly
hot. But even if some komatiites are some 200°C hotter than present
day MORBs and depleted picritic melts, this would not prove a plume
origin. Significant lateral variations of temperature are implied by
geophysical data and modelling (e.g. Kaula, W.M., Minimal upper
mantle temperature variations consistent with observed heat flow and
plate velocities, J. Geophys. Res., 88, 10,323-10,332,
1983). Magma temperatures of 50-100°C hotter than the MORB
mean are well within the range of expected plate tectonic variations.
I believe that the highest inferred temperature for Gorgona komatiites
is still within the range expected for the upper mantle. Substantial
cooling since the Archean is expected and this helps to
resolve the so-called “missing heat-source paradox”.
You have also uncovered a new paradox. Why are there
so few oceanic plateaus trapped in the interiors (or edges) of old continents?
A current (but minority) trend in mantle geodynamics
and magma genesis is to relate features attributed to plumes from the
core-mantle-boundary (CMB) to shallow and plate tectonic processes instead.
The idea that komatiites are related to subduction, rather than the
CMB, is a particularly dramatic reversal of conventional wisdom.
last updated 9th July, 2004
|
|
|